Architectured Materials
The Architectured Materials Group aims at developing novel materials that fill desirable white spaces in the Ashby diagrams. We investigate fundamental processes of small scale plasticity under extreme conditions and multiscale toughening mechanisms in complex hierarchical materials. We translate this knowledge into the design of novel metamaterials with hierarchical architecture and tailored microstructure that allow us to combine usually mutually exclusive properties, e.g. high strength and toughness with a low density. To achieve this, we actively develop nanomechanical instruments and methods and closely integrate experimental and modeling approaches.
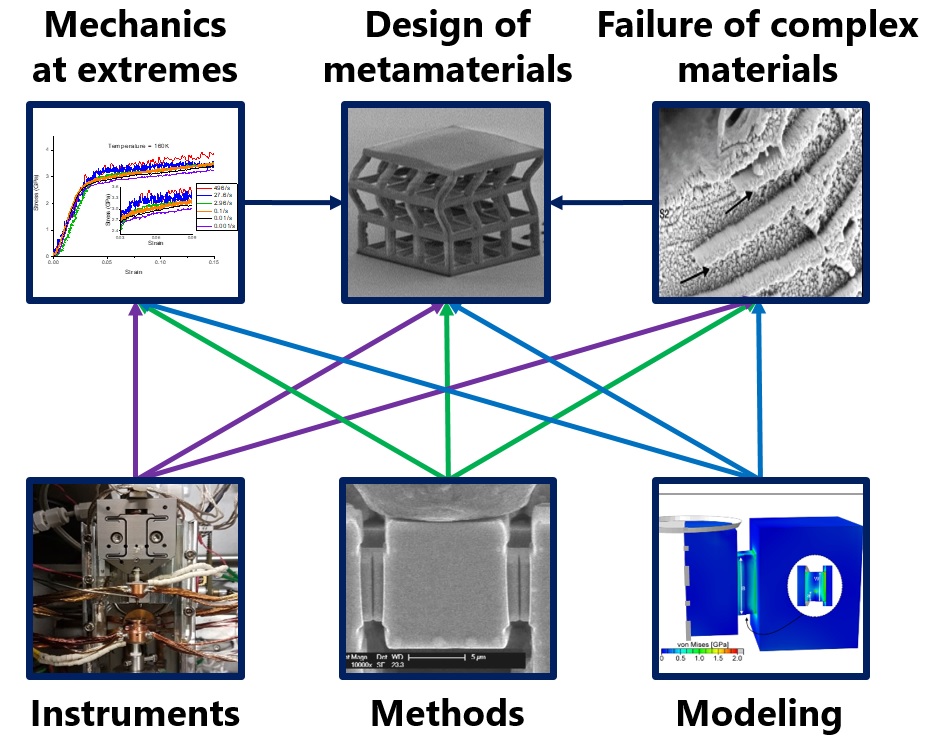
Fundamental processes of plasticity and failure in microscale components
A potential pathway towards high specific strength materials is to make use of both extrinsic and intrinsic size effects in the design of mechanical metamaterials. We investigate the rate limiting plastic processes at the nanoscale and the effect of grain and component size on the mechanical behaviour through a combination of thermal activation analysis at low temperatures and high strain rates, microstructural analysis, and computational modeling. A thorough understanding of the fundamental deformation mechanisms enables us to find pathways for influencing the mechanical behavior of the material through microstructural design. To achieve this, we push the limits of what can be measured at the microscale today through active instrument and method development, e.g. for mode-dependent fracture, tensile, cryogenic temperature, or high strain rate experiments. (Materials & Design 2022, Materials & Design 2020, JMR 2019, Nano Letters 2019, FFEMS 2018)
Multiscale failure of hierarchical materials
Furthermore, we study the failure of complex materials such as hierarchical biological nanocomposites (e.g. bone, wood, teeth) or nanostructured thin films to identify how these materials manage to combine toughness and strength with a light weight through multiscale toughening mechanisms. We assess the influence of their complex microstructure, i.e. the role of interfaces, defects, and material inhomogeneity, on their macroscopic failure. Combining challenging mechanical experiments, microstructural analysis, and mechanical modeling spanning from the nano- to the macroscale allows us to investigate and model the underlying physical processes in these materials at the relevant length scales. (Acta Biomaterialia 2021, Acta Biomaterialia 2020, Acta Biomaterialia 2017, Nature Materials 2014)
Design, synthesis, and characterization of hierarchical metamaterials
Based on the gained knowledge on fundamental processes at the nanoscale and multiscale toughening mechanisms, we develop novel materials that feature advantageous combinations of properties such as a high specific strength and toughness through simulation-assisted design. This is achieved by making use of size effects, microstructural design, and hierarchical architecture through a combination of microscale additive manufacturing techniques like two photon lithography, electrodeposition, and atomic layer deposition, to prepare materials that are light, strong and damage resistant and fill desirable white spaces in the Ashby diagrams. (Materials & Design 2022, Materials & Design 2020, Adv. Mater. Technol. 2018)
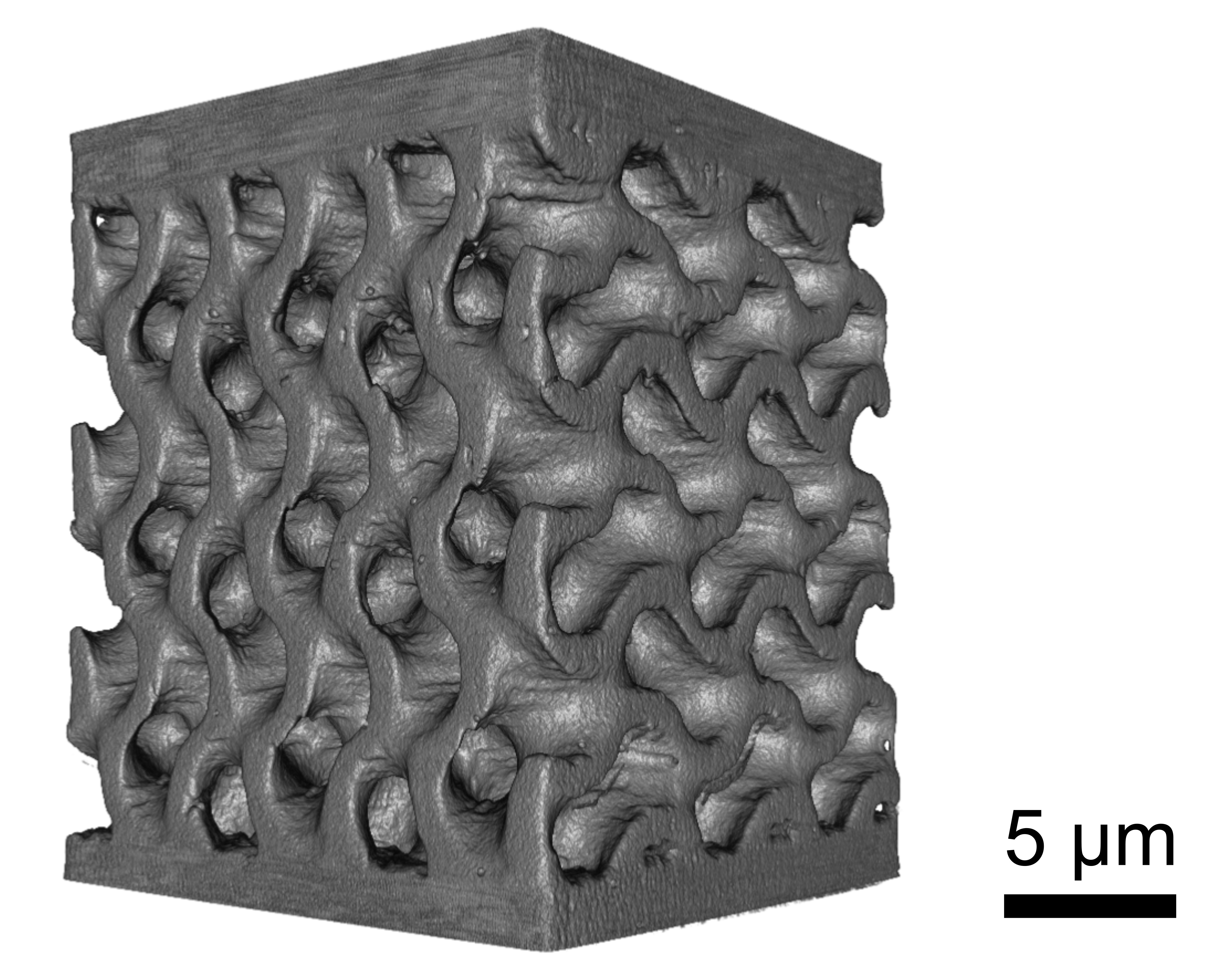
Projects:
Computing human head elastic waves for bone anchored hearing aids
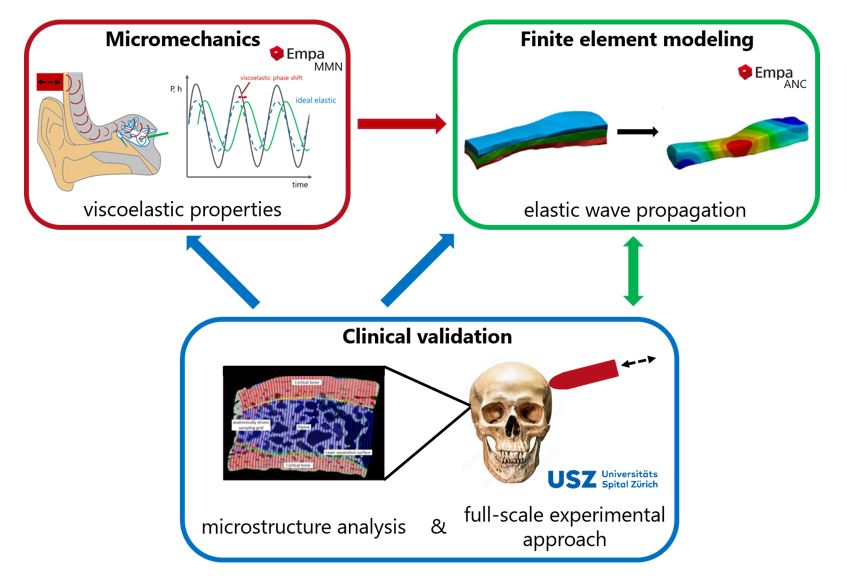
The aim of this multidisciplinary project is to create a realistically fast, practical, and validated model of the acoustic response in the cochlea resulting from bone conduction hearing aids stimulation. Cutting-edge engineering methods, both numerical (Empa, Lab of Acoustic/ Noise control – ANC) and experimental (Empa, Lab of Mechanics of Materials and Nanostructures – MMN) will be combined with high-level clinical validation (University Hospital Zürich) experiments. Apart from the direct application to create better and more reliable hearing aids, and to understand the influence of individual skull geometries, the project will lead to several fundamental results.
Firstly, very few studies have investigated the viscoelastic properties of bone in the audible range due to limited testing capabilities. We will therefore develop a novel indentation device which is able to determine the material's dynamic response in a wide frequency range. Second, the application of advanced finite element techniques (material homogenization, fluid-structure interaction, model order reduction) for hearing can be transferred to other biomechanical problems, or more general engineering applications. The input data and validation measurements provided by the clinical partner are a unique crosslink ensuring the future use of the project's outcome.
Efficient development of compositionally complex coatings
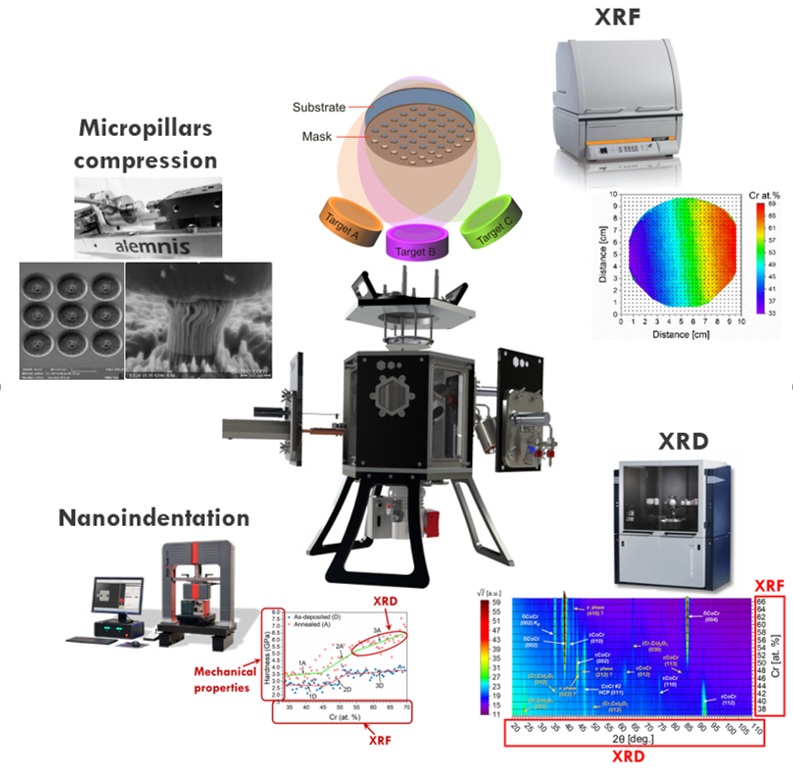
The EU Horizon 2020 research and innovation action project FORGE objective is to develop a set of cost-effective highly protective coatings, based on novel Compositionally Complex Materials (CCMs) to provide the required hardness, chemical stability and gas barrier properties for challenging industrial applications. As part of the consortium, we utilize high throughput processes for efficient material discovery based on thin film combinatorial material libraries. These are synthesized by physical vapor deposition and analyzed in terms of their composition, microstructure, and mechanical performance under various boundary conditions to identify process-composition-structure-property relationships. This allows the effective screening of material libraries to identify materials of interest for specific appli-cations like improved chemical or wear resistance.
Microscale 3D printing by local electrodeposition
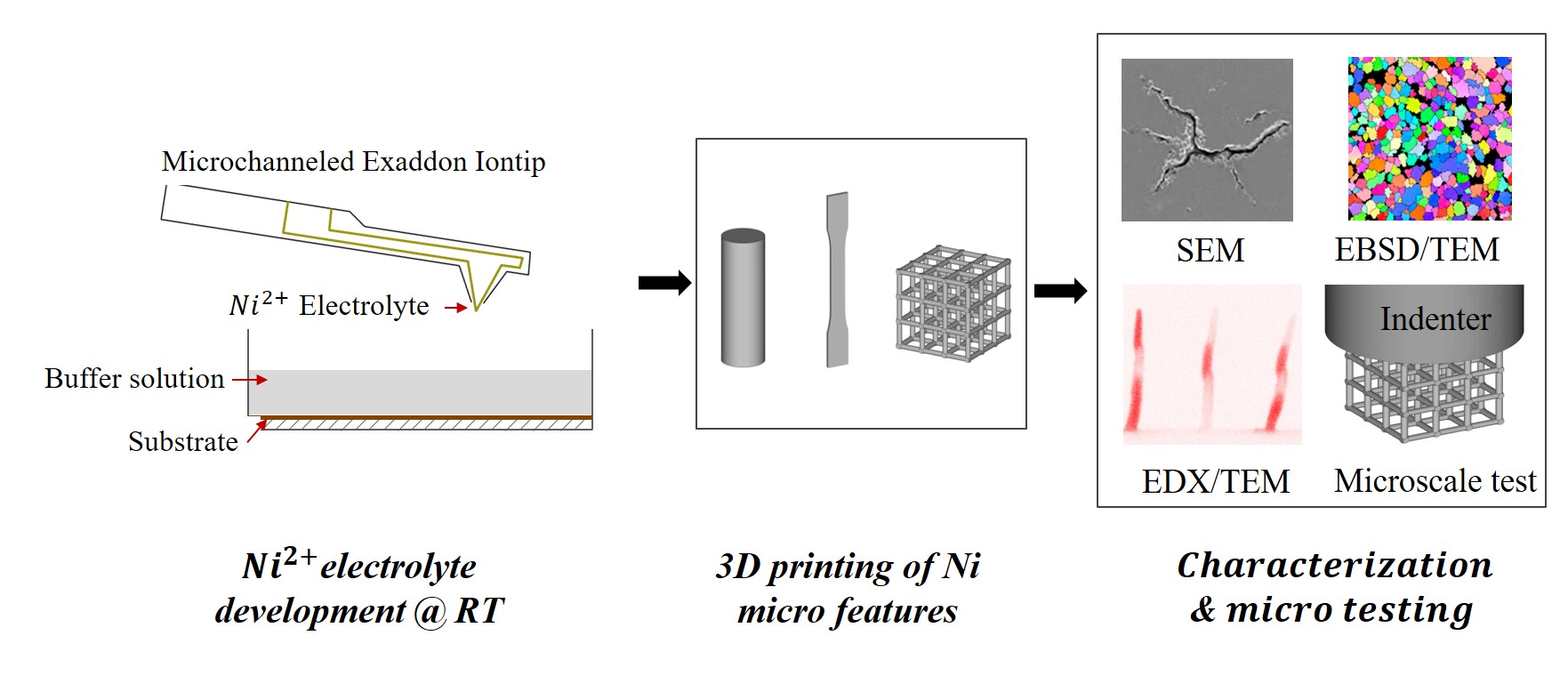
The aim of the Innosuisse innovation project NIPRINT (3D Microprinting of Nickel) is to develop a process that allows printing of microscale nickel components by local electrodeposition, using the CERES µAM print system manufactured by Exaddon AG (Zürich, Switzerland). The project includes bath chemis-try optimization, hardware and software development, as well as deposit characterization. Microstructures deposited at different printing parameters are characterized using different analytical techniques, such as SEM, EDX, XRD, EBSD and TEM, while the mechanical properties, such as Young's modulus, strength, ductility and creep behavior, are investigated by micromechanical testing at extreme conditions.
In situ high-speed nanomechanical testing inside the scanning electron microscope
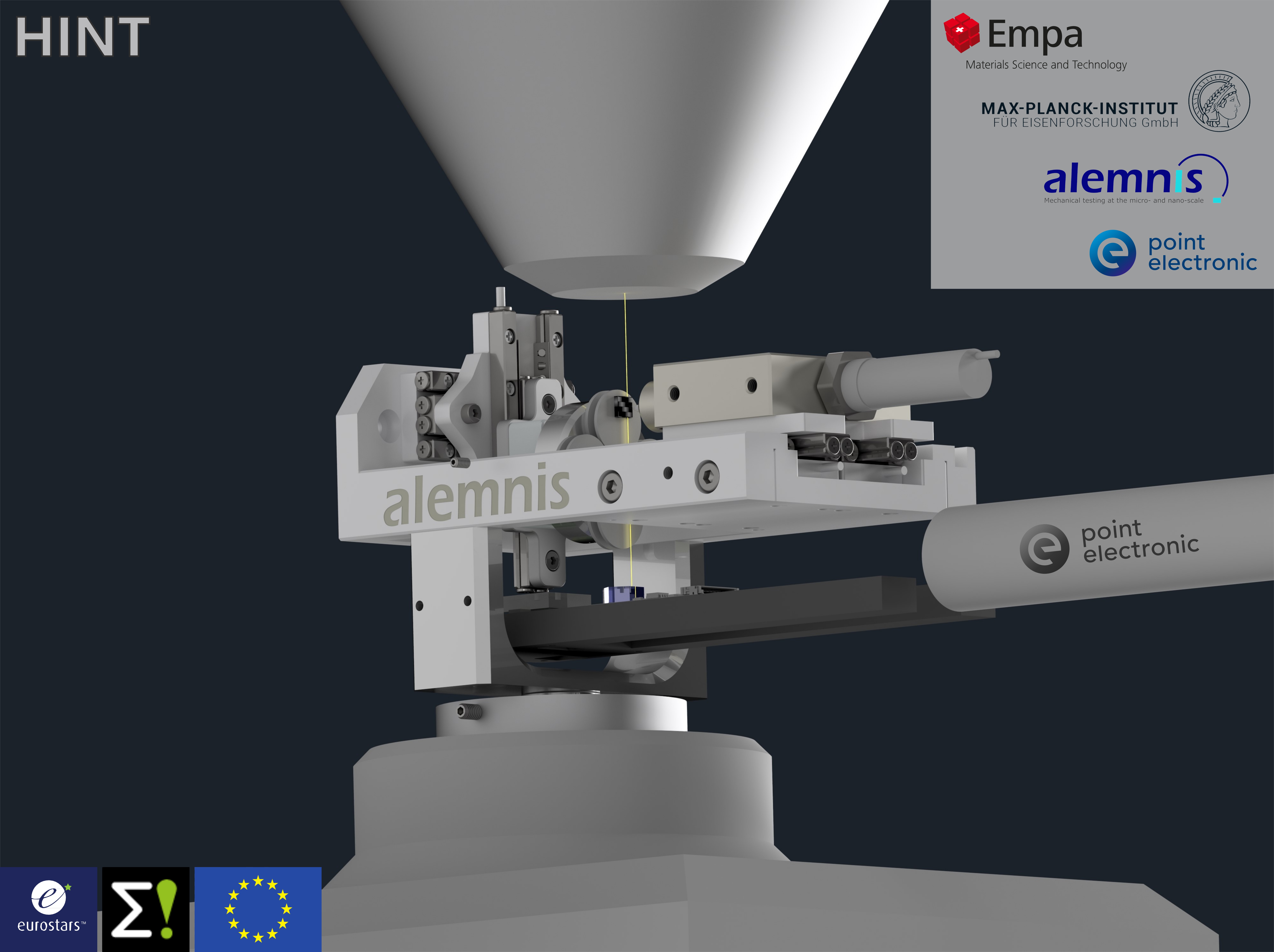
HINT (High-speed in situ nanomechanical tester) is a project financed by the Eureka Eurostars funding scheme and a collaborative effort of four project partners from academia and industry. Empa (Thun, Switzerland), the Max Planck Institute for Iron Research (Düsseldorf, Germany), point electronic GmbH (Halle, Germany) and Alemnis AG (Thun, Switzerland) are working together with the aim of developing a novel stand alone in situ device that combines high-resolution nanomechanical testing with high-speed imaging. These features will be coupled with nanoscale characterisation at extreme conditions including strain rates of up to 1000 s-1. The new device will allow the dynamic in situ study of structural changes of samples at the nanometre length scale during mechanical testing. The new insights will give invaluable feedback on small scale structures and the optimisation of their architecture and design.
Developing mechanically tunable optical metamaterials
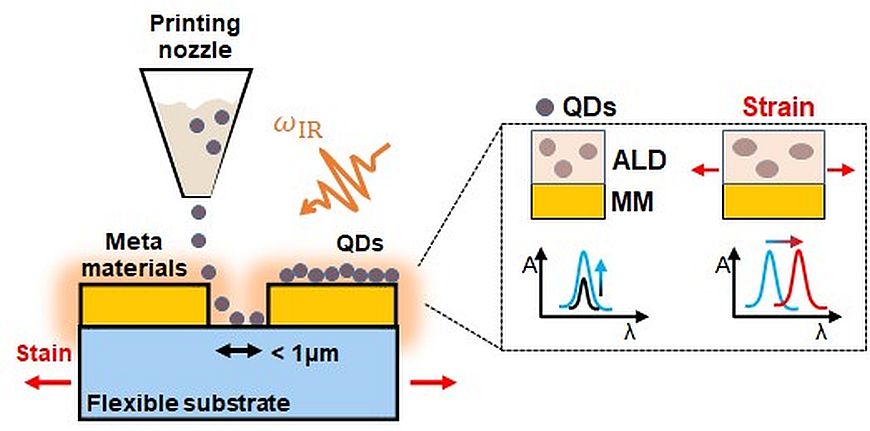
The focus of the project MetaQD is to lay the foundations for infrared nanostructured metamaterials-based detectors that combine nano-elements using directed self-assembly, with the goal of developing advanced photonic devices with improved optical performance that are mechanically tuneable for added functionality for sensing applications. Colloidal quantum dots (QDs) have emerged as promising nanomaterials for broadband detection as their sensitivity depends on size and can be tuned from visible to mid-infrared (MIR) range. However, their absorption cross-section is quite low, thus limiting their potential. A possible solution are optical resonant nanostructures: squeezing light into small mode vol-umes enhances light-matter interactions dramatically. Nanostructured metamaterials can be used to enhance light absorption in QDs resulting in responsivity up to 5 μm within the midwave infrared win-dow, where disturbance from atmospheric influence can be avoided. This has the potential to increase device sensitivity and adds functionality allowing sustainable research. The proposed project will lay the foundation for the development of next generation nano-opto-mechanical devices for various applications.
Zinc metamaterials as an architected coating for biomedical implants

The BioCoaTED project's main aim is to design an architected zinc (Zn) microlattice with tunable geometrical and structural configurations offering unique properties that eliminate emerging orthopedic implant complications. Zn is a promising candidate for biomedical uses due to its biodegradation ability, bioactive properties, and antimicrobial behavior. As a coating material, Zn is expected to present much better mechanical performance than low-strength polymer coating and better plasticity than ceramic-based coatings. The individual goals of the project assume optimization of the fabrication parameters to produce high-quality porous structures with controllable geometry, investigation of intrinsic and extrinsic features' effects on the biocompatibility, biodegradation, and mechanical behavior of Zn microlattices, and verification how the thin zinc oxide layer deposited on the 3D structure can modify these properties. The project's expected outcome assumes coating fabrication on the orthopedic implant surface via periodical repetition of the design microlattice.
Comprehensive research, including microscopic analysis, micromechanical testing, biodegradation, and biological properties evaluation, will deliver fundamental information on structure-properties relations in designed Zn microlattices and provide valuable knowledge on their biomedical potential. Producing an optimized microlattice that may be used for implant coating will benefit patients by reducing the risk of inflammation and implant rejection, as recovery time, which in turn accelerates tissue regeneration, reduces surgery-related stress, and increases the healthcare system's cost efficiency.
Microscale additive manufacturing of fused silica meta-materials for high specific strength and toughness
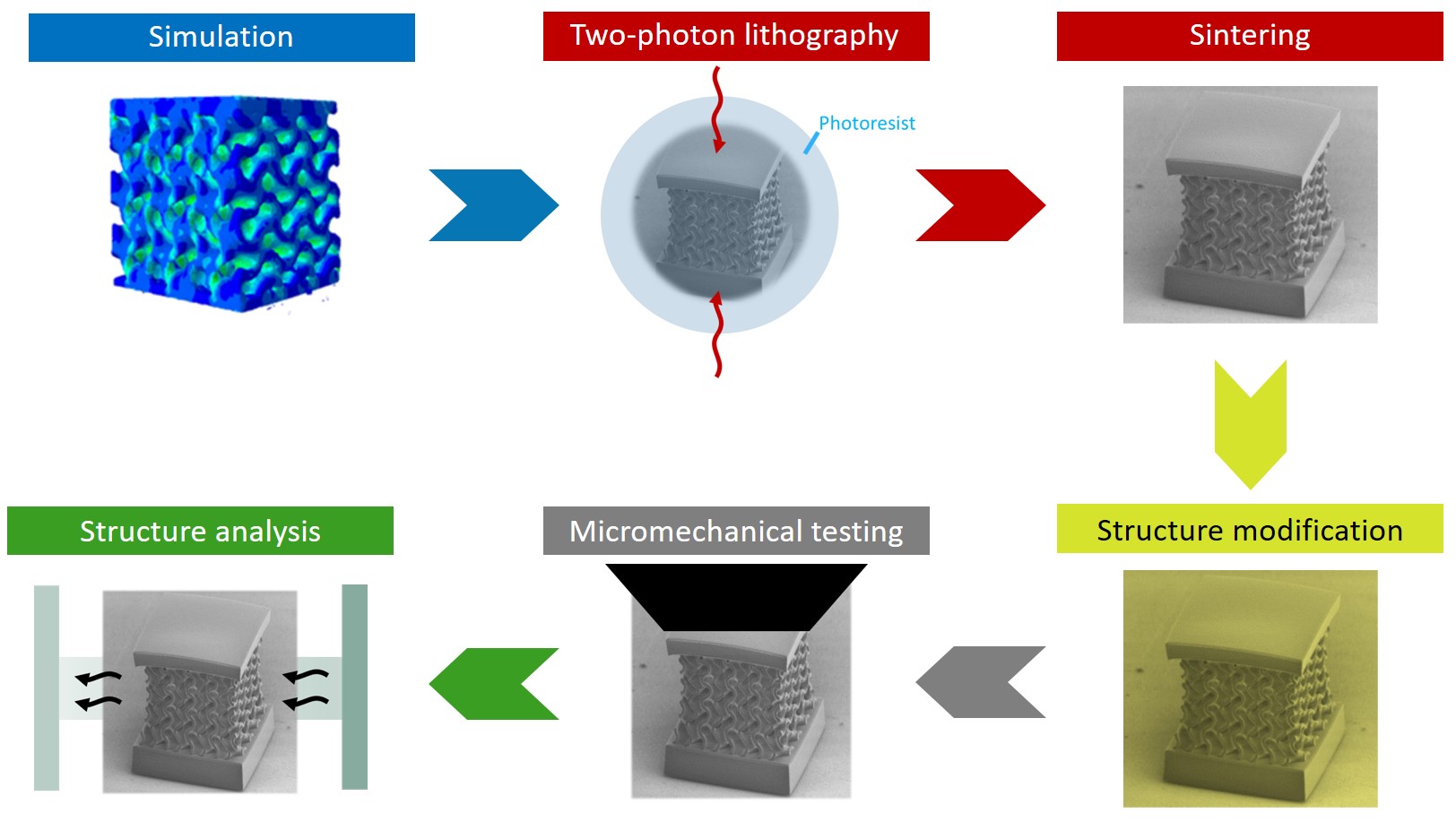
Recent advances in microscale additive manufacturing enable the printing of complex micrometer-sized silica glass structures using two-photon lithography. Manufacturing structures with sub-micron sized features would allow producing nanoscale metamaterials, whose properties exceed those of their conventional counterparts due to mechanical size effects.
In this project financed by the Swiss National Science Foundation in the context of the COST action MecaNano, nanoscale deformation and failure mechanisms of fused silica based metamaterials with micron sized features will be elucidated under different loading modes. Micromechanical experiments at varying temperature and strain rate will be carried out. Modifications to glass chemistry and structure will be achieved by a combination of thin film deposition and heat treatment and process-structure-property relationships will be identified. The aim is to design, synthesize and characterize micron-scale metamaterials with superior specific strength and toughness. Moreover, the knowledge gained will be used to identify and validate physics-based material models that allow designing nanoscale metamaterials using a simulation-based approach. Finally, the influence of imperfections and defects in the printed structures will be assessed by means of high resolution imaging.
The project will thus enable an improved fundamental understanding of the nanoscale mechanisms rendering fused silica an especially attractive material for microscale devices and establish design rules and novel synthesis routes for preparing complex architectures. These results will open up diverse potential applications, e.g. in shock-absorbing and thermal barrier coatings, scaffolds for tissue engineering, or mechanically resilient nano-optical devices and sensors.
Additive micromanufacturing of miniaturized strong and stiff probes
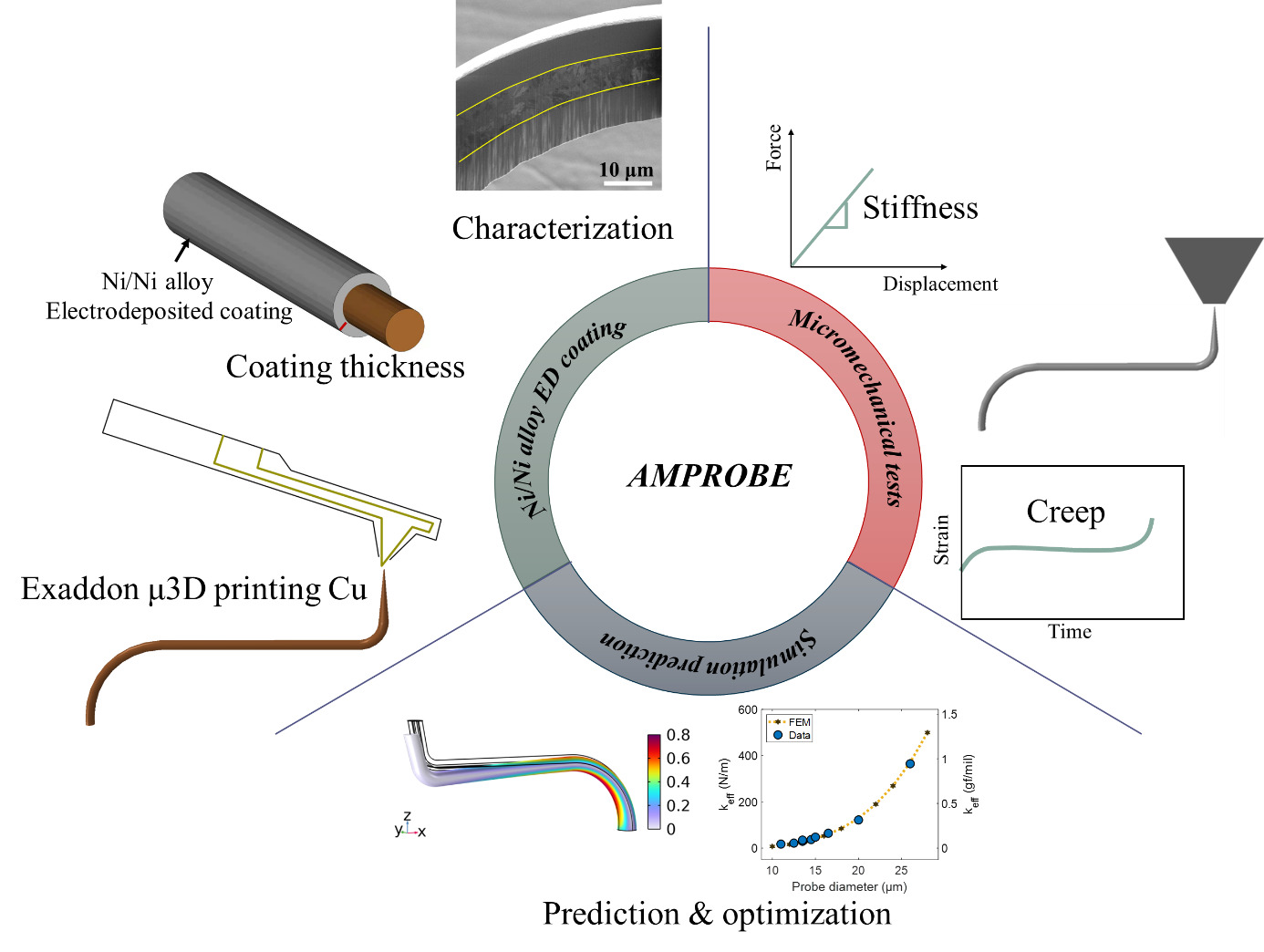
The miniaturization of daily devices demands a robust fabrication of tiny but mechanical strong and stable components. Under the AMPROBE project, we will combine the microscale additive manufacturing technique from EXADDON and electrodeposition coating to produce core-shell bending probes with high stiffness and low creep rates. The core material will be pure copper because of a high printing speed and high application potential in microelectronics. For the coating shell, nickel or nickel based alloy will be applied owing to their superior stiffness compared to copper, consecutively characterized with respect to chemical composition and microstructure. Micromechanical tests will be performed on these probes in order to evaluate the stiffness and creep rates, further validating the simulation. An optimization is desired on shell thickness and alloy content to reach a good combination of mechanical properties, with the assistance of experimentally validated simulation and modelling.
Improving individual fracture risk prediction in osteogenesis imperfecta: an integrated multi-omics, materials science, and computational modeling approach
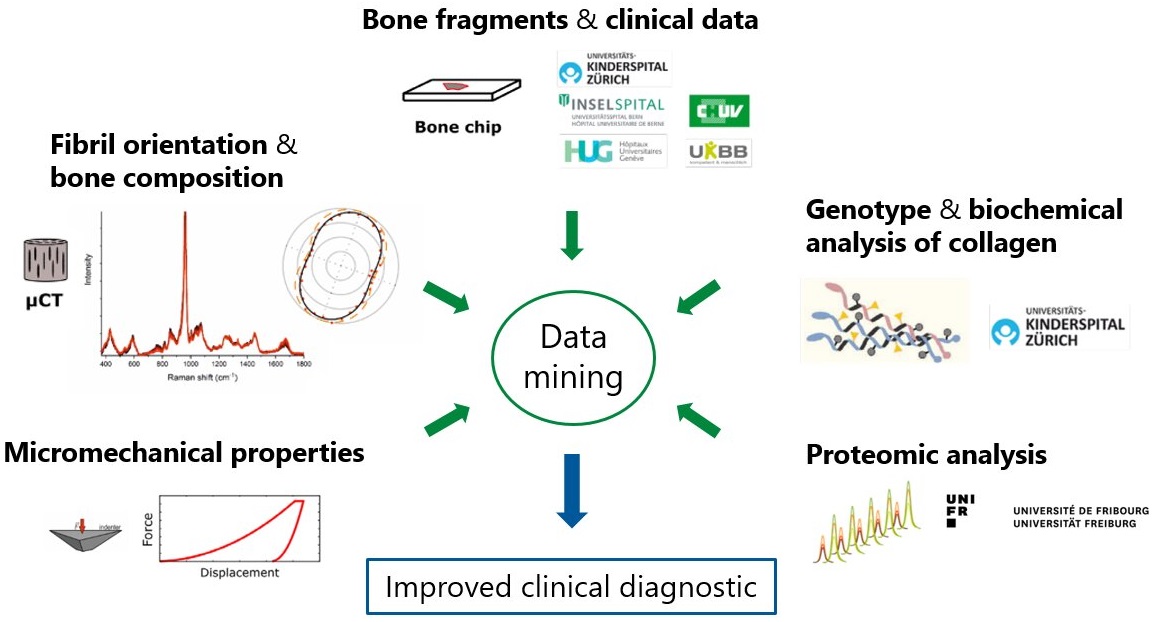
Osteogenesis imperfecta (OI) is a severe genetic disease that affects the quality and quantity of bone tissue and can severely impair patient's quality of life. In particular, increased bone fragility in individuals with OI leads to an increased fracture rate after minimal trauma. Due to a wide phenotypic variation and insufficient understanding of the effects of this pathology on bone ECM quality and properties, diagnosis and treatment of OI are currently limited.
The main aim of this project, which is funded by the ETH Strategic Focus Area Personalized Health and Related Technologies, is to combine clinical data, bone microscale analysis methods and machine learning to improve current diagnostic tools used in a clinical setting. Collaboration with 5 partner hospitals (Insel, CHUV, HUG, UKBB, Kispi) allows obtaining relevant patient data and OI bone samples. Recent advances in microscale characterization allow us to study structure-property relationships at the bone ECM level in the presence of different OI types and treatments. This information is combined with biochemical, proteotype and clinical information. Using data mining techniques, it is investigated if the integration of the multimodal dataset may be used to reliably predict patient-specific OI type and bone fracture risk.
This research has the potential to shed light on the underlying pathological changes of OI on the tissue-scale and their relationship with standard clinical data. This in turn will make possible to find prospective clinically accessible biomarkers that, correlating with the microscale effects, are potentially useful for an improved OI diagnosis and fracture risk prediction.